Early Atomic Theory
Once classification systems were established, scientists
naturally turned to understanding the theory behind such relationships.
Actually they discovered that this had been attempted several times in
the past. In the last 100 years, this theory has become quite involved
and represents a major field of chemistry.
Leucippus, born ca. 500 BCE, and his pupil, Democritus,
born ca. 460 BCE, are credited with postulating the theory of Atoms and
Void. Democritus expanded upon Leucippus' original theory and theorized
on many of its detailed applications like perception. Leucippus believed
that the formative substance of the universe was the One, an infinite,
all encompassing, motionless mass that contained no empty space, void.
Leucippus theorized that this theory could not be correct; since our senses
tell us that there is motion. He postulated that void, the absence
of all anything that exists, is necessary for motion. He also postulated
that an infinite number of particles, atoms, forms what exists and that
the dissolution of these particles leads to the destruction or death of
that object. The use of atoms solves the problem with the Eleatic
principle that nothing that truly is can change or come into existence
or perish. Only the forms that the atoms comprise are changing; the
atoms themselves are changeless and complete within themselves.
The theory on void and atoms developed by the Atomists
is developed for us by Aristotle in Metaphysics. The shape and existence
of all things are determined by void and atoms through the differences
in shape, position and arrangement of the atoms and the proportion of void
in the substance. They believed that there was an infinite number
of atoms that were indivisible and impassable. These atoms do not
contain any void, making them indivisible because void between bodies is
necessary to break the bodies apart. The absence of void makes the
atoms incredible compact, weighing a lot proportional to their size.
Since all material is made of atoms, the more dense a material is the less
void it contains. An example of this theory is the beliefs about
fire; philosophers believed that fire was a state of matter, like water.
The Atomists believed that fire contained atoms separated by a large area
of void; therefore, fire rises out of the material that produces it.
In
1672 Newton published his first scientific paper on light and colour
in the Philosophical Transactions of the Royal Society. The
paper was generally well received but some objected to Newton's attempt
to prove, by experiment alone, that light consists of the motion of
small particles rather than waves. However, perhaps because
of Newton's already high reputation, his corpuscular theory reigned
until the wave theory was revived in the 19th century. Newton's
greatest achievement was his work in physics and celestial mechanics,
which culminated in the theory of universal gravitation. By 1666 Newton
had early versions of his three laws of motion. He had also
discovered the law giving the centrifugal force on a body moving uniformly
in a circular path. However he did not have a correct understanding
of the mechanics of circular motion. Newton analyzed the motion
of bodies in resisting and non-resisting media under the action of
centripetal forces. The results were applied to orbiting bodies, projectiles,
pendulums, and free-fall near the Earth.
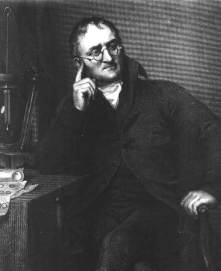
This led Dalton to propose that all bodies of sensible magnitude,
whether liquid or solid, are constituted of a vast number of extremely
small particles, or atoms of matter bound together by a force of attraction,
which is more or less powerful according to circumstances, and which as
it endeavours to prevent their separation, is very properly called in that
view, attraction of cohesion; but as it collects them from a dispersed
state (as from steam into water) it is called, attraction of aggregation,
or more simply affinity. If some of the particles of water were heavier
than others, if a parcel of the liquid on any occasion were constituted
principally of these heavier particles, it must be supposed to affect the
specific gravity of the mass, a circumstance not known. Similar observations
may be made on other substances. Therefore we may conclude that the
ultimate particles of all homogeneous bodies are perfectly alike in weight,
figure, and etc. In other words, every particle of water is like every
other particle of water; every particle of hydrogen is like every other
particle of hydrogen..
The concept of the atom as a quantifiable object
had arrived. A little over 100 years later, Thomson proposed the
first
structure
of the atom. It took this long to verify the existence of negative
and positive components of matter. Thomson regarded the as containing
a large number of smaller bodies or corpuscles which are equal to each
other. In the normal atom, the individual corpuscles behave like
negative ions, yet when they are assembled in a neutral atom the negative
effect is balanced by something which causes the space through which the
corpuscles are spread to act as if it had a charge of positive electricity
equal in amount to the sum of the negative charges of the corpuscles.
The detached corpuscles behave like negative ions, each carrying a constant
negative charge while the part of the atom left behind behaves like a positive
ion with the unit positive charge and a mass large compared with that of
the negative ion. However, not everyone is convinced this is the
right answer such as Savante Arrhenius (the 1903 Nobel Prize winner in
Chemistry). Sometimes the Thomson Model is presented incorrectly
.
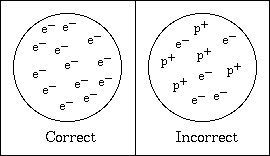
New
Zealander-English physicist Rutherford is best known for devising the names
alpha, beta, and gamma rays to classify various forms of "rays" which
were poorly understood at his time (alpha and beta rays are particle beams,
while gamma rays are a form of high-energy electromagnetic radiation ).
He deflected alpha rays with both electric and magnetic fields in 1903.
He also observed that the intensity of radioactivity fell off with time,
and named the halving time the "half-life." In 1906, his students
Geiger and Marsden conducted the classic gold foil alpha particle scattering
experiment which showed large deflections for a small fraction of incident
particles. This led Rutherford to proposed that the atom was "nuclear."
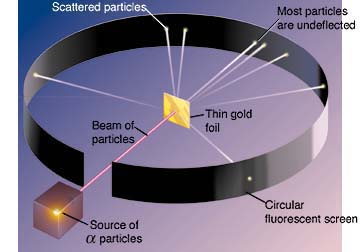
Rutherford suggested that the simplest possible rays
must be those obtained by hydrogen and that these must be the fundamental
positively charged particle, which he dubbed the proton in 1914.
In 1917, he passed alpha particles through a gas of nitrogen and occasionally
observed scintillation of hydrogen impacting on his screen. He concluded
that the alpha particles were knocking protons out of the nitrogen atoms,
and thus that he had made the first observation of nuclear reactions.
According to Rutherford's theory, electrons could
orbit the nucleus at any distance. When the electrons circle round
the nucleus, they are constantly changing their direction. According
to classical electrodynamics (which deals with the motion of electrons),
such electrons which either constantly change their direction or their
velocity or both should continuously emit radiation. While doing
so, they should lose energy, and thus spiral into the nucleus. This
means every atom is unstable, quite contrary to our observation.
Furthermore, the Rutherford atom could not account for some observations
concerning the behaviour of certain gases. These gases at low pressure
emit light in a set of discrete bands of the electromagnetic spectrum.
This is quite different from the radiation emitted by solids, which is
spread evenly across the electromagnetic spectrum. The radiation
emissions of these gases were important because they showed that at least
under some circumstances, the orbits of the electrons could not be at just
any distance from the nucleus, but were confined to discrete distances
(or energy states).
Bohr received his doctorate in physics from the University
of Copenhagen in 1911. He then traveled to Manchester, England
to study under British physicist Ernest Rutherford. In 1913 Bohr
published a theory about the structure of the atom based on an earlier
theory of Rutherford's. Rutherford had shown that the atom consisted
of a positively charged nucleus, with negatively charged electrons in orbit
around it. Bohr expanded upon this theory by proposing that electrons
travel only in certain successively larger orbits. He suggested that
the outer orbits could hold more electrons than the inner ones, and that
these outer orbits determine the atom's chemical properties. Bohr
also described the way atoms emit radiation by suggesting that when an
electron jumps from an outer orbit to an inner one, that it emits light.
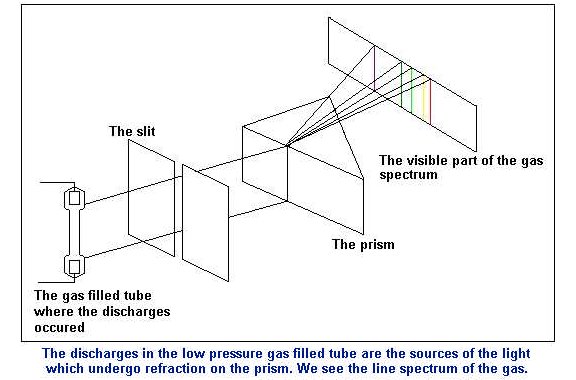
He proved this idea by separating light emitted from
heated sample of gases into spectral colours demonstrating the energy fields
electrons travel within. His work has yielded spectra charts showing
the possible excitation states of electrons within atoms.
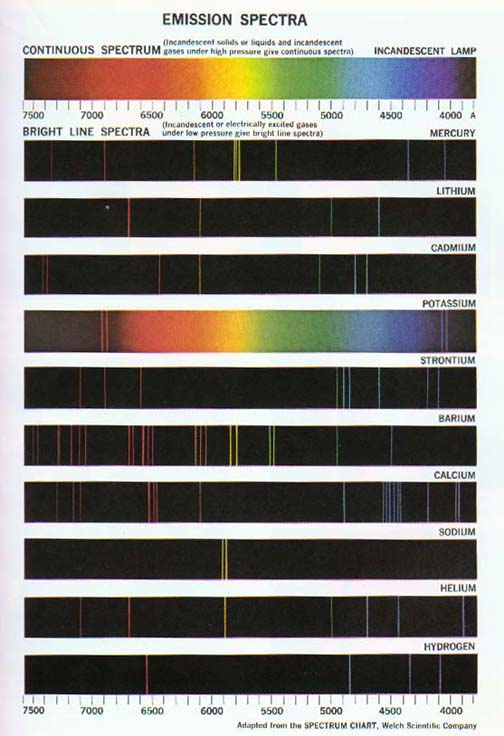
Until 1932, the atom was known to consist of a positively
charged nucleus surrounded by enough negatively charged electrons to make
the atom electrically neutral. Most of the atom was empty space,
with its mass concentrated in a tiny nucleus. The nucleus was thought
to contain both protons and electrons because the proton (otherwise known
as the hydrogen ion, H+) was the lightest known nucleus and because electrons
were emitted by the nucleus in beta decay. In addition to the beta
particles, certain radioactive nuclei emitted positively charged alpha
particles and neutral gamma radiation. Twelve years earlier, Lord
Ernest Rutherford, a pioneer in atomic structure, had postulated the existence
of a neutral particle, with the approximate mass of a proton, that could
result from the capture of an electron by a proton. This postulation stimulated
a search for the particle. However, its electrical neutrality complicated
the search because almost all experimental techniques of this period measured
charged particles. In 1928, a German physicist, Walter Bothe, and
his student, Herbert Becker, took the initial step in the search.
They bombarded beryllium with alpha particles emitted from polonium and
found that it gave off a penetrating, electrically neutral radiation, which
they interpreted to be high-energy gamma photons. In 1932, Irene
Joliot-Curie, one of Madame Curie’s daughters, and her husband, Frederic
Joliot-Curie, decided to use their strong polonium alpha source to further
investigate Bothe’s penetrating radiation. They found that this radiation
ejected protons from a paraffin target. This discovery was amazing because
photons have no mass. However, the Joliot-Curies interpreted the
results as the action of photons on the hydrogen atoms in paraffin.
They used the analogy of the Compton Effect, in which photons impinging
on a metal surface eject electrons. The trouble was that the electron
was 1,836 times lighter than the proton and, therefore, recoiled much more
easily than the heavier proton after a collision with a gamma photon.
We now know that gamma photons do not have enough energy to eject protons
from paraffin.
When James Chadwick reported to Lord Rutherford on
the Joliot-Curies’ results, Lord Rutherford exclaimed, "I do not
believe it!" Chadwick immediately repeated the experiments at the Cavendish
Laboratory in Cambridge, England. He not only bombarded the hydrogen
atoms in paraffin with the beryllium emissions, but also used helium, nitrogen,
and other elements as targets. By comparing the energies of recoiling
charged particles from different targets, he proved that the beryllium
emissions contained a neutral component with a mass approximately equal
to that of the proton. He called it the neutron in a paper published
in the February 17, 1932, issue of Nature. In 1935, Sir James Chadwick
received the Nobel Prize in physics for this work. It is interesting
to note that the Joliot-Curies’ misinterpretation of their results cost
them the Nobel Prize. (Not to worry; in 1935, they received the Nobel Prize
in chemistry for their discovery of artificial radioactivity.)
The nuclear atom had arrived.
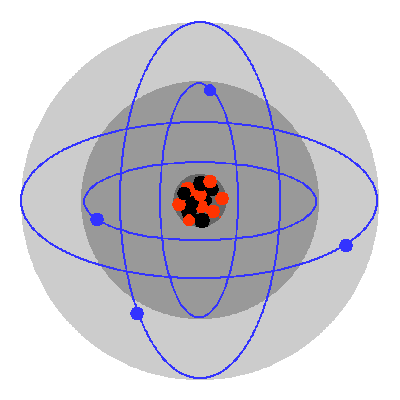
Isotopic Abundance
With the discovery of neutrons, discrepancies in atomic masses of pure samples of elements made sense. Elements can exist as isotopes. Chemical identity and properties remain constant since the number of electrons and protons remain the same, but nuclear properties and mass can differ because the same element can have more than one atomic version due to a differing number of neutrons. Some of these isotopes proved to be quite valuable to society so efforts were made to identify and separate specific isotopes of an element. Technology had to be invented to accomplish this and although, it has improved considerably over time, the basic design remains the same. The devise utilized is called the mass spectroscope. To understand how it works, consider this simplified model.
1. |
The actual amount of sample must be very small. Often you see this stage in television shows when they show a small needle injecting a sample of blood or paint or drug into a machine. |
2. |
Converting the sample into a gas is essential since gas particles lack any significant inter-particle attraction for each other which means each particle can be influenced and act independently. This will allow the particles to ultimately be separated from each other. |
3. |
As soon as the sample is heated to a gas, the electron field generates an intense area of negative charge by moving electron through wires which will force electrons to fly off the gas particles of the sample. If more charge is applied, more electrons can be knocked off and this can allow scientists some control as it will determine how positively charged the particles become which will later influence how fast they move through the magnetic field which ultimately alters their degree of separation. |
4. |
Immediately after becoming positively charged particles, the magnetic slits will activate to generate the appropriate magnetic pole to attract the positive ions. The number of magnets is actually much more numerous than than shown here and they are electromagnets which allows scientists another way to alter the ultimate speed and hence separation of ions later. |
5. |
The electromagnetic quickly alter their poles to sequentially attract-repel-attract the positive ions ensuring they only move forward and also further accelerates the motion of the positively charged ions. They function in a peristaltic-like fashion such that the plate ahead attracts while the plate behind repels and as soon as the positive ions moves through the hole in the plate, that plate switches to repulsion while the next forward plate becomes attractive. |
6. |
If there were no secondary electromagnetic, the heavier isotopes would slow sooner and fall to the bottom of the device, while the lighter isotopes with their greater momentum would continue to move further forward. However, this is not practical because the entire length of the tube would need to be coated with the expensive detection plate and the tube would need to be quite long (they are moving quite fast). Imagine if you had a machine shoot out a collection of ping-pong balls, golf-balls and metal ball-bearings of the same size moving at the same speed. The heavy ball bearings would slow first, followed by the intermediate massed golf balls and finally the light ping-pong balls would slow and fall to the ground.
Typically, the tube is bent and lined with additional electromagnets. If the electromagnets are complete around the tube and an attractive charge (pole) is induced in them, the positive isotopes will be pulled to the outside the tube to varying degrees based on mass. This will pull the lighter isotopes more to the outside to hit the detection plate in circular rings of decreasing mass with the heaviest isotopes concentrated in the center because since they are traveling at the same speed, more mass = less impact. Imagine if you blow on the group of moving balls above. The light ping-pong balls would deflect the most since momentum is a function of both speed and mass and they have less mass so changing their momentum is easiest. Of course, if the electromagnets were set up for repulsion, the lighter isotopes would move more to the center, but this would force more clumping with the heavier isotopes so that would not be a good idea.
If the only bottom side of the electromagnets is turned on to repulsion, the lighter isotopes would move to the top of beam (as depicted). Attraction would not be ideal as this would lead to more clumping at the bottom. |
7. |
The detection plate is actually the most sophisticated piece of technology in this device and requires a computer. It can count the number of hits received per area and using math determine the percentage of the total sample that must be a specific mass. This is how both the specific isotopic mass based on amount of spread amongst the groups of particles (a mass ratio) and the isotopic abundance based on the percentage of hits in that area. |
Typical mass spectroscope data for isotopic abundance is displayed as follows:
However, it is also routinely used to verify the identity of a specific chemical within an impure sample.
As you can see from the graphs, the output is in terms of charge (supplied to make the positive ions) versus mass of the separated atoms. This is the critical math needed to understand how this device works; that is, is the mass-to-charge ratio.
This is simply the isotope mass divided by the charge applied by the device and it indicates the degree of isotopic separation on the deflection plate. The smaller the ratio, the greater the separation. A fraction of 1, the highest ratio means all the isotopes hit in the same spot which is not ideal. This means not enough charge was supplied to spread out the masses from each other. This ratio really implies degree of clumping. A fraction of 0 means the isotopes were so spread out, there were no repetitive hits at all which is also not ideal. Neither of this situations happen, but often scientists work to improve separation or lower the ratio to improve identification of distinct isotopes. Improving separation can be achieved by using lower massed isotopes, but typically isotope presence is determined randomly as we are analyzing an unknown sample (why would you need to isolate a sample that must have been previously isolated for identification anyway). Or it can be achieved by applying more charge to initially repel electrons off the gaseous sample to make the ions more positive. This will ultimately make the positive ions move faster and spread further apart from each other based on mass in that lighter isotopes will move further away. Hopefully this allows enough separation to clearly identify the isotopes present.
Therefore, the mass spectrometer can be adjusted to improve isotopic separation. The operator can increase the electron bombardment on the sample to create more positive charge on the gas ions which would lower the mass-to-charge ratio causing greater separation of isotopes which causes isotopes to spread apart more according to mass. The operator could use additional electromagnets near the end to pull more on the lighter positive particles as they pass by because they will more momentum as they travel and this will increase the distance between them and the heavier particles. The operator could use additional initial electromagnets to accelerate the positive particles more to allow more distance between massed particles since they will have longer to diverge from each other. Finally, a longer spectrometer could be used (not practical) so there is longer time for the particles to move away from each other because the lighter particles will not lose as much momentum as they travel and this will increase the distance between them and the heavier particles allowing easier detection of differences between them.
The Bohr-Rutherford Atom
The Bohr-Rutherford model of the atom consists of
four principles:
-
Electrons assume only certain orbits around the nucleus. These orbits are
stable and called "stationary" orbits.
-
Each orbit has an energy associated with it. For example the orbit closest
to the nucleus has an energy E1, the next closest E2 and so on.
-
Light is emitted when an electron jumps from a higher orbit to a lower
orbit and absorbed when it jumps from a lower to higher orbit.
-
The energy and frequency of light emitted or absorbed is given by the difference
between the two orbit energies.
Bohr's model only worked with hydrogen atoms, yet many
went on to determine more of the nuclear atom's structure. It was
found that the maximum number of electrons confined to a particular energy
level was determined by the distance from the nucleus. Thus the first
energy level could only hold 2 electrons as it was very close to the nucleus
and strong forces of repulsion would prevent more electrons from occupying
this region. It turned out that the maximum number of electrons within
an energy shell was equated to 2n2 where n is the principal
quantum number or shell number. Thus the further one gets from the
nucleus, the more electrons can exist within a shell.
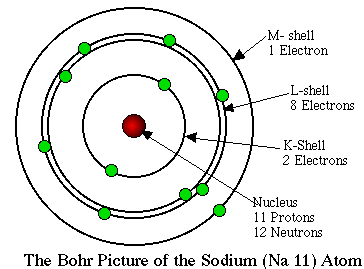
Based on the Bohr-Rutherford atom, it was now possible
to make predictions regarding the properties of elements within the periodic
table based on the interaction of indepedently changing forces of attraction due to increasing atomic number (protons) and repulsion due to electrons surrounding the nucleus. Essentially, if number of electrons change within the same level, changes in electron-electron repulsion are only slight so changing proton number will be significant. However, if new levels of electrons are added, the electron-electron repulsion becomes significant and changing proton number will be nullified. Generally, when the force of attraction due to protons is greater than the force of repulsion due to electrons, atoms tend to gain electrons and if the force of attraction due to protons is less than the force of repulsion due to electrons, atoms tend lose electron.
As a result, certain characteristics follow trends going across rows or
down columns in the periodic table. Moving to the right across a
single row or period of the table, any one electron experiences more of
an attraction to the nucleus because the nucleus contains more positive
charges but electron added remain in the same level. Therefore, the electrons are more tightly bound to the nucleus
toward the right of the row because the force of attraction is increasing faster than the force of repulsion. Thus, atoms decreases the size (radius) moving from left to
right.
Traveling down a column means adding more substantially more electrons and protons; however, the electrons added create new shells of electrons. Although both forces of attraction due to protons and repulsion due to electrons increase dramatically, the force of repulsion increases to a larger extent and so the size of the atoms increases.
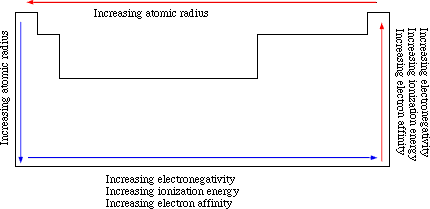
This interplay of the forces of attraction and repulsion account for the changes in the ability to remove electrons from gaseous atoms or ionization energy. Likewise, these forces explain electron affinity which is the
energy released when a gaseous atom receives an additional
electron. Both of these energies will be natural changes if the result is enhanced stability (typically as in removal of electrons from metals and addition of electrons to non-metals), but if the atom becomes less stable then the process may not result in a lasting change. That, is, if energy is used to remove an electron from an atom that then becomes less stable, the situation will likely revert when allowed as seen typically with non-metals and noble gases. Or, if addition of an electron does not result in an atom becoming more stable which would be measured by a release in energy, then the outcome will not persist and the electron will soon be ejected from the atom as would typically be the case for metals and noble-gases. Both of these unstable conditions can be maintained, but only if energy is continuously expended. This is easier to force to happen in terms of ionization energy because it is simple enough to just continually use energy to maintain the unstable condition. However, in the case of electron affinity, this is harder to maintain because electrons can more easily fly off if repulsion is too great. This condition is actually called negative electron affinity since energy would be consumed instead of released as expected and is quite atypical. Trends in negative electron affinity are not as linear since many other factors can affect such a subtle change.
When an electron is removed or added it causes a
rebalancing of forces which affects atomic properties.
For example is an electron is removed, the remaining electrons experience slightly less eletron-electron repulsion for the same amount of force of attraction from protons (did not change in number) or if an entire level is lost as result, there will be a substantial decrease in electron-electron repulsion. This will subsequently make it harder to remove further electrons.
In fact if there are remaining electrons within an energy level, they will
be pulled closer to the nucleus making the + ion smaller than the neutral
atom. The diminished electron-electron repulsion further allows the
electron-reduced shell to move closer to the nucleus. If the electron
is the only electron within that shell, the atom will lose an entire level
making the + ion considerably smaller than the neutral atom. The
successive ionization energies are often measured to help elucidate the
structure of an atom. Whenever, there is a substantial jump in ionization
energy, the corresponding electron removed must be coming from an energy
shell that is closer to the nucleus. Thus, this electron is receiving
a stronger nuclear force of attraction and is harder to remove.
When an electron is added to a neutral atom, the
increased electron-electron repulsion forces all the electrons further
apart since the force of attraction was constant. As a result - ions tend to be larger than their
neutral atoms. Quite often the first electron added is
an exothermic process, but subsequent electron addition is endothermic
as electron-electron repulsion becomes substantial and the nuclear force
is too weak to freely accept the additional extra electron.
The elements of the periodic table can be classified
into three main groups: metals, semi-metals or metalloids, and nonmetals.
In addition, certain columns of the periodic table have recognizable features.
A dividing line exists in the periodic table beginning between boron and
aluminum and proceeding stepwise down to the right (i.e., passing next
between aluminum and silicon and then between silicon and germanium).
Metals are those elements found to the left of this line, nonmetals are
on the right of this line, with the semi-metals or metalloids lying adjacent
to the line. Metals are generally malleable (easily shaped), ductile
(easily pulled into wire), good conductors, and lustrous. Nonmetals
are generally brittle and poor conductors. Their other characteristics
vary more than those of metals. Semi-metals or metalloids possess
characteristics of both metals and nonmetals. The members of this
group are the least uniform in character. Semi-metals may act as either
metals or nonmetals, depending on the situation. In other words,
in certain compounds, semi-metals may bond with nonmetals (acting as metals),
while in others they may bond with metals (acting as nonmetals).
The alkali metals are the elements found in group IA of the periodic table.
All of these metals have one valence electron and are thus very reactive.
They tend to have a softer consistency. The alkaline earth metals
are the elements in group IIA of the periodic table. These elements have
two valence electrons and have positive electron affinities. The
transition metals are the elements located in the B groups of the periodic
table. Transition metals may have several oxidation states; that
is, they give up their electrons fairly easily and have several to lose.
Since the d orbitals (more on this later) are filling in this section of
the periodic table, the d electrons are not in complete shells or subs-hells
and may be removed. In addition, the outer two valence electrons (from
a higher energy shell) may be removed. Since the electrons are so
easily removed, the transition metals are extremely good conductors.
These elements also have high melting and boiling points. They may form
complexes with water when dissolved in solution. The halogens
are the elements in group VIIA of the periodic table. These elements only
need one additional electron to complete their outer shells. As a
result, they form bonds very easily. The halogens with lower atomic numbers
are diatomic gases at room temperature, while those with higher atomic
numbers are liquids and solids. Finally, the noble gases are the last column
on the right of the periodic table. These elements do not readily
form compounds and are gases at room temperature.
The Quantum Atom
Some irregularities in the trends were starting to
cause concern. Why does nitrogen have a higher stability than predicted?
Why do group II metals resist accepting an additional electron? Max
Planck (1858-1947) theorized that light beams were made of photons that
are equivalent to particles of wave motion. These discoveries created
new concepts. When these concepts and discoveries are integrated, new ideas
emerge. The result is a quantum theory, named perhaps from the discrete
nature of energy levels in microscopic systems. This theory gives
good interpretations of the phenomena of the atomic and subatomic world
and could account for these irregularities.
At the sub-atomic scale, there is no boundary between
particles and waves. In fact, both particles and wave properties
must be considered simultaneously for a system. Furthermore, when
coupled with the theory of relativity developed by Einstein, there is no
boundary between material and energy. Energy and mass are equivalent,
and they can convert into each other. Further support for a new model
came from more careful spectra analysis revealing that the line spectra
of many gases could be further divided into groups. It appeared that
within an energy level, there were groups of electrons acting in sub-groups.
This led to the development of states of electrons
represented by wave functions. Each wave function has a set of numbers,
called quantum numbers. Electronic states, represented by wave functions,
in an atom are called atomic orbitals. Since we use quantum numbers to
describe them, atomic orbitals are labeled by quantum numbers, such as
2s orbital. Each atomic orbital accommodates two electrons due to
electron spin.
The principal quantum number is defined as the number
of the energy shell to which an electron belongs. Next it is important
to identify the sub-shells that exist within that energy level. the
lowest sub-shell is always s which has been found to only hold 1
pair of oppositely spinning electrons. Since the first energy level
only has 2 electrons, it only has one sub-level. The next level again
must start with the lowest energy sub-level, s, followed by 3 pairs of
electrons found to have similar energy signatures. This sub-level
is called p. The second level can only hold 8 electrons which corresponds
to the presence of s and p (2 + 6). The next level holds 18 electrons
(2 from s + 6 from p and now 10 from a new higher energy sub-level d)
The fourth level holds 32 electrons (2 from s, 6 from p, 10 from d and
now 14 from f).
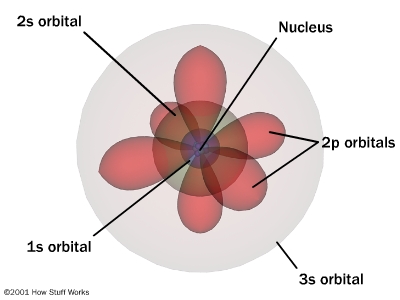
Since electrons occur in pairs with opposing spins,
each of these sub-levels has a unique shape. s is a sphere because
it only has 2 electrons in it. p has 3 pairs of electrons spread
out as far as possible creating 3 bi-lobed structures. Similarly
d and f have unique shapes and orientations as well.
An orbital may be considered as a
region in space to which electrons have access. Heisenberg's uncertainty
principle states that one cannot simultaneously determine with certainty
both the position and the momentum of an electron. So, an orbital
may be represented as a region where there is a given probability
of finding an electron at any given time. The diagram below
shows how the probability density, (P), of a 1s electron varies with
its distance (r) from the nucleus. This so-called electron density
of an electron in a 1s state is the same in all directions.
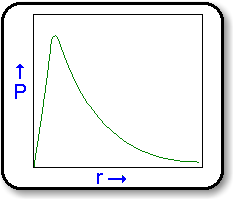
Similarly, probability distributions can be determined
for other sub-shells.
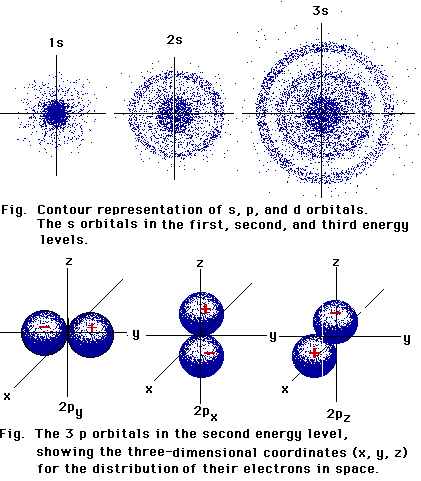
p-Orbitals consist of electron clouds which look
like the diagram on the right, with a node at the nucleus of the atom.
There are three such orbitals, oriented at right angles to one another,
as shown in the diagram below:
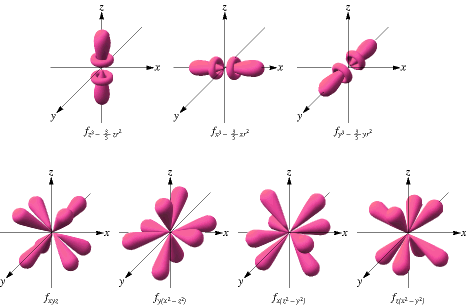
The actual order of filling these sub-levels does
not go sequentially as one might anticipate because some of the sub-levels
have higher energy values than predicted.
Consider lithium. This element has 3 electrons.
We would thus begin by placing two electrons in the 1s ground state, or
lowest energy, orbital. These two electrons would have opposite magnetic
spin quantum numbers. We would then place the third electron in the next
highest energy level orbital - the 2s orbital:
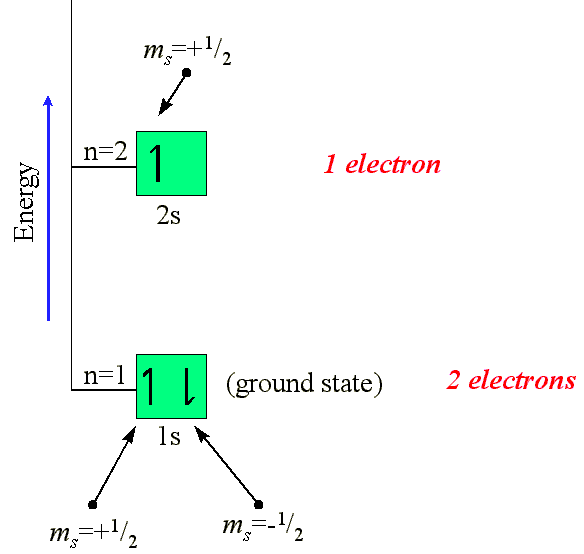
The arrows indicate the value or direction of the
magnetic spin. The description of the occupation of the orbitals
would be described in the following way: 1s22s1.
Electrons having opposite spins are said to be "paired" electrons, as with
the electrons occupying the Li 1s orbital. Likewise, the single electron
in the 2s orbital (for Li) is said to be "unpaired". The notation
can be broken down as follows:
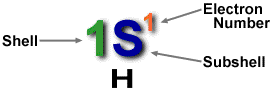
We can continue filling in the electronic configuration
of atoms following this pattern.
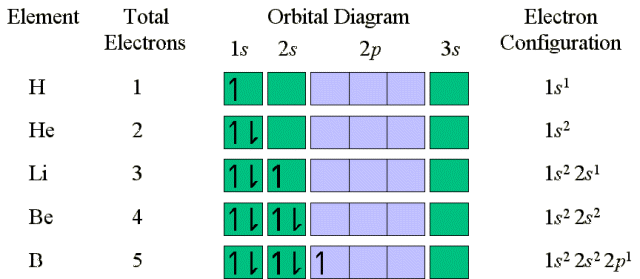
The two electrons in He represent the complete filling
of the first electronic shell. Thus, the electrons in He are in a very
stable configuration. For Boron (5 electrons) the 5th electron must
be placed in a 2p orbital because the 2s orbital is filled. Because the
2p orbitals are equal energy, it doesn't matter which 2p orbital is filled.
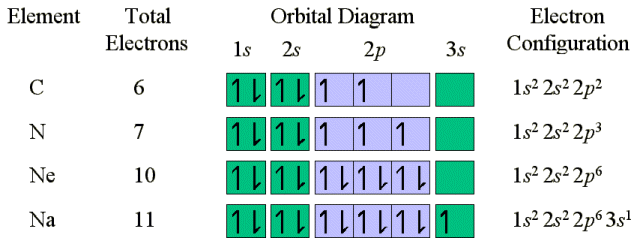
The second 2p electron in Carbon is placed in another
2p orbital, but with the same spin as the first 2p electron because we
follow Hund's rule which states that for degenerate orbitals, the lowest
energy is attained when the number of electrons with the same spin is maximized.
Since electrons repel each other, by occupying different orbitals the electrons
remain as far as possible from one another. A carbon atom in its
lowest energy (ground state) has two unpaired electrons
Ne has filled up the n=2 shell, and has a stable electronic configuration.
Electronic configurations can also be written in a short hand which references
the last completed orbital shell (i.e. all orbitals with the same principle
quantum number 'n' have been filled). The electronic configuration
of Na can be written as [Ne]3s1. The electronic configuration
of Li can be written as [He]2s1. The electrons in the
stable (Noble gas) configuration are termed the core electrons. The
electrons in the outer shell (beyond the stable core) are called the valence
electrons. The noble gas Argon (18 electrons) marks the end of the
row started by Sodium.

The next electron to be placed does not occupy 3d
as it has been found to be higher in energy than 4s.
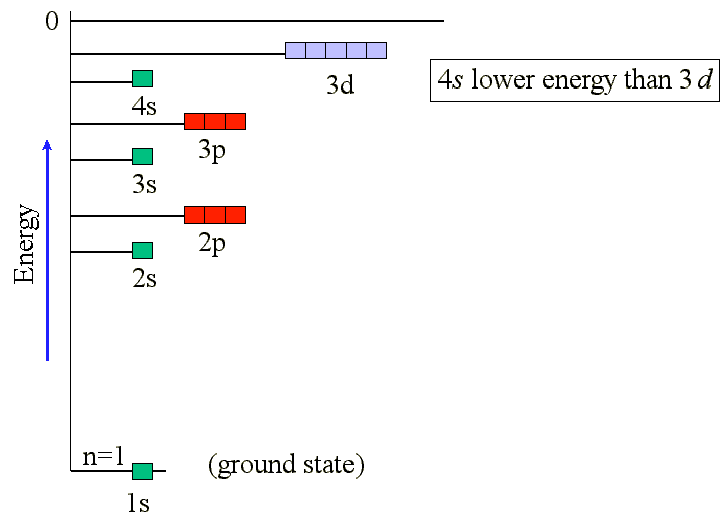
The actual sequence of sub-shell filling can be determined
by using the following chart. The order represents moving from the
lowest energy sub-shell to the highest energy sub-shell.
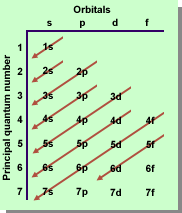
When atoms are arranged according to the highest
energy sub-shell filled, the following pattern or blocks can be identified.
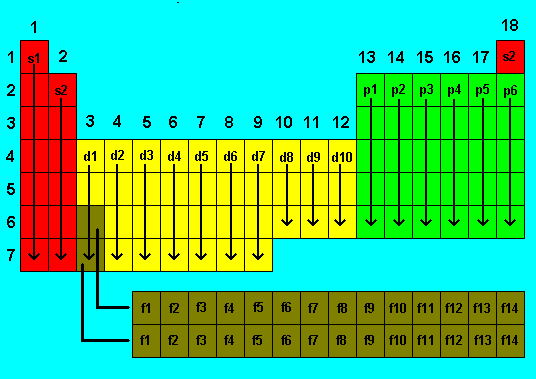
Although the order of orbital filling given in class
is a good "rule of thumb" there are many exceptions. There are a
number of reasons for this. Every time a new element is formed a
new nucleus is made, and that affects the relative energies of the orbitals.
The f-elements (the lanthanides and actinides) vary tremendously in this
respect, so their electronic configurations often vary from the "rule of
thumb". The graph of ionization energy versus atomic number is not
a perfect line because there are exceptions to the rules that are easily
explained.
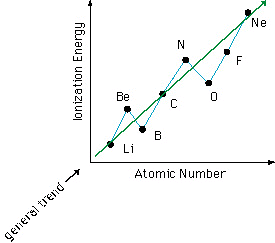
Filled and half-filled subs-hells show a small increase
in stability in the same way that filled shells show increased stability.
So, when trying to remove an electron from one of these filled or half-filled
subs-hells, a slightly higher ionization energy is found.
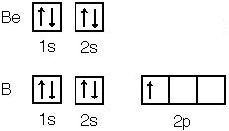
It's harder to ionize an electron from beryllium
than boron because beryllium has a filled "s" sub-shell. Nitrogen
also has a half-filled "2p" sub-shell so it is harder to ionize an electron
from nitrogen than oxygen.
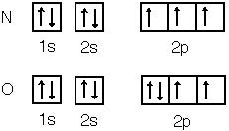
Some atoms will rearrange their electronic configuration
in order to achieve this half stability. Consider chromium.
Vanadium has one less electron and its electronic configuration is [Ar]3d34s2
but chromium is [Ar]3d54s1 which was not the anticipated
[Ar]3d44s2. Chromium has changed its electron
distribution to achieve higher stability by promoting an electron from
a lower energy sub-shell to a higher sub-shell. This requires energy,
but more energy is released due to the enhanced stability. This can
only happen when one electron can move up one sub-shell. Half stability
comes in many forms: s1, s2 p0,
s2 p3, s2d5, s2d10.
It is tempting to remember the pattern, but better to remember the reasoning.
Half stability occurs when electrons can be spread out to minimize electron-electron
repulsion within the same sub-shell. Thus have single electrons in
each orientation (p3) or completely filled orientations
(d10) provides an atom with magnetic stability. This must
be considered when writing electronic configuration or trying to understand
periodic trends.
References
http://www.perseus.tufts.edu/GreekScience/Students/Marc/short_paper.html
http://www-gap.dcs.st-and.ac.uk/~history/Mathematicians/Newton.html
http://web.lemoyne.edu/~giunta/dalton.html
http://dbhs.wvusd.k12.ca.us/webdocs/AtomicStructure/Thomson-Model-Intro.html
http://cwx.prenhall.com/bookbind/pubbooks/blb/chapter2/medialib/blb0202/bl02fg09.jpg
http://chemcases.com/nuclear/nc-01.htm
http://www.iun.edu/~cpanhd/C101webnotes/modern-atomic-theory/Bohr-model.html
http://www.cbu.edu/~mcondren/bohr.gif
http://www.scientia.org/cadonline/Chemistry/periodictable/periodtrend.ASP
http://www.science.uwaterloo.ca/~cchieh/cact/c120/quantum.html
http://hmchemdemo.clt.binghamton.edu/zumdahl/docs/chemistry/07atomstructure/f0717.gif
http://wine1.sb.fsu.edu/chm1045/notes/Struct/EConfig/Struct08.htm
http://www.epcc.edu/faculty/victors/elconfig.htm
http://www.physchem.co.za/Atomic/Electron%20Configuration.htm
http://www.webchem.net/notes/atomic_structure/electron_configuration.htm
http://www.uwm.edu/~dwbent/Chem102/WkOct25.htm
http://www.chemistry.ohio-state.edu/~grandinetti/teaching/Chem121/lectures/periodic%20trends/ionization_energy.html