Bonding
Chemical bonds form to lower the energy of the system,
the components of the system become more stable through the formation of
bonds. If we examine the periodic table, we find that the elements
in Group VIII (or 18), helium, neon, argon and so on, are particularly
stable, so much so that they were once labeled the "inert gases". We now
know that these elements are not inert, indeed xenon forms a range of compounds,
but, nevertheless, they are very stable (although now we refer to these
elements as the noble gases). This stability is the result of their electronic
configuration, they have a full valence shell of electrons (s2,
p6) and this imparts stability. G. N. Lewis (1916) suggested
that bonds (covalent) formed to enable elements to attain this "noble gas
configuration". While some of Lewis' predictions have since been proven
incorrect (he suggested that electrons occupy cube-shaped orbitals), his
work established the basis of what is known today about chemical bonding.
We now know that there are two main types of chemical bonding, ionic bonding
and covalent bonding.
In ionic bonding, electrons are completely transferred
from one atom to another. In the process of either losing or gaining
negatively charged electrons, the reacting atoms form ions. The oppositely
charged ions are attracted to each other by electrostatic forces which
are the basis of the ionic bond. For example, during the reaction
of sodium with chlorine, sodium loses its one valence electron to chlorine
resulting in a positively charged sodium ion and a negatively charged chlorine
ion.
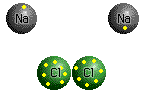
Notice that when sodium loses its one valence electron
it gets smaller in size, while chlorine grows larger when it gains an additional
valence electron. This is typical of the relative sizes of ions to
atoms, positive ions tend to be smaller than their parent atoms while negative
ions tend to be larger than their parent. After the reaction takes
place, the charged Na+ and Cl- ions are held
together by electrostatic forces, thus forming an ionic bond. Notice
also that sodium now has 10 electrons similar to neon while chorine now
has 36 electrons similar to Argon. Hence both atoms have attained
the electronic configuration of a noble gas when they became ions.
As a result a higher level of stability has been achieved.
Ionic compounds share many features in common:
- Ionic bonds form between metals and non-metals,
- In naming simple ionic compounds, the metal is always first, the non-metal
second (i.e.. sodium chloride),
- Ionic compounds dissolve easily in water and other polar solvents,
- In solution, ionic compounds easily conduct electricity,
- Ionic compounds tend to form crystalline solids with high melting temperatures.

This last feature, the fact that ionic compounds are solids, results from
the intermolecular forces (forces between molecules) in ionic solids.
If we consider a solid crystal of sodium chloride, the solid is made up
of many positively charged sodium ions (pictured at right as small gray
spheres) and an equal number of negatively charged chlorine ions (green
spheres). Due to the interaction of the charged ions, the sodium
and chlorine ions are arranged in an alternating fashion as demonstrated
in the schematic at right. Each sodium ion is attracted equally to
all of its neighboring chlorine ions, and likewise for the chlorine to
sodium attraction. The concept of a single molecule becomes blurred
in ionic crystals as the solid exists as one, continuous system.
Forces between molecules are comparable to the forces within the molecule,
and ionic compounds tend to form crystal solids with high melting points
as a result.
The second major type of atomic bonding occurs when
atoms share electrons. As opposed to ionic bonding in which a complete
transfer of electrons occurs, covalent bonding occurs when two (or more)
elements share electrons. Covalent bonding occurs because the atoms
in the compound have a similar tendency for electrons (generally to gain
electrons). This most commonly occurs when two non-metals bond together.
Because both of the non-metals will tend to gain electrons, the elements
involved will share electrons in an effort to fill their valence shells.
A good example of a covalent bond is that which occurs between two hydrogen
atoms. Atoms of hydrogen (H) have one valence electron in their first
electron shell. Since the capacity of this shell is two electrons,
each hydrogen atom will 'want' to pick up a second electron. In an
effort to pick up a second electron, hydrogen atoms will react with nearby
hydrogen (H) atoms to form the compound H2. Because the
hydrogen compound is a combination of equally matched atoms, the atoms
will share each others single electron, forming one covalent bond.
In this way, both atoms share the stability of a full valence shell.
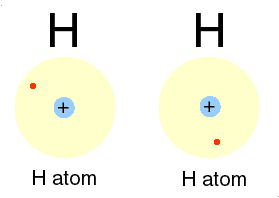
Unlike ionic compounds, covalent molecules exist
as true molecules. Because electrons are shared in covalent molecules,
no full ionic charges are formed. Thus covalent molecules are not
strongly attracted to one another. As a result, covalent molecules
move about freely and tend to exist as liquids or gases at room temperature.
For every pair of electrons shared between two atoms,
a single covalent bond is formed. Some atoms can share multiple pairs
of electrons, forming multiple covalent bonds. For example, oxygen
(which has six valence electrons) needs two electrons to complete its valence
shell. When two oxygen atoms form the compound O2, they
share two pairs of electrons, forming two covalent bonds.
Resonance
Sometimes, a single Lewis structure
does not adequately represent the true structure of a molecule. Consider
the carbonate ion, CO32- . Carbon (C) has four
valence electrons and oxygen (O) has six valence electrons. Carbon
is the central atom, the three oxygens are bound to it and electrons are
added to fulfill the octets of the outer atoms.

All the available electrons
have been used but carbon is electron deficient - it only has six electrons
around it. So, we share a non-bonding electron pair on an oxygen with the
carbon to create a double bond and thereby fulfill carbon's octet.
becomes 
But two other possible configurations are possible:
becomes
becomes 
We can sum this up as:
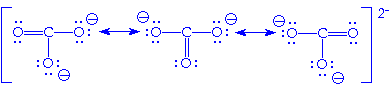
From a quantum perspective, it is wrong to think
that these forms are oscillating back and forth when in reality they all
exist equally at the same time. This may be be challenging to think
about since you are used to thinking of electrons as discrete points which
due to a rather Newtonian way of thinking of the world.
These structures are created as a result of attempting
to form the most stable arrangement of electrons. Clearly the task
will become harder if more than eight electrons are involved in bonding.
Orbitals
Since electrons are constantly
in motion and do not represent discrete points, how can we properly visualize
the bonding of electrons. We need to appreciate that electron position
can only be predicted based on probability theory. S orbitals only
contain two electrons, each traveling in different directions from each
other. As a result there is little electron-electron interaction.
As a result the s orbital forms a perfect sphere as there is an equal probability
of finding an electron in every direction around the nucleus of the atom.
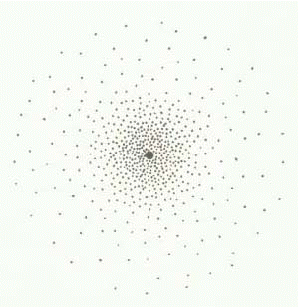
However, it is much easier to draw this as:
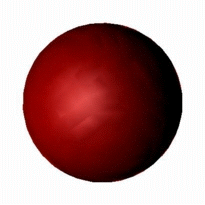
The p orbital is more complex since p can hold six
electrons, but each orbital can only hold 2 electrons. Thus there
are three equal orbitals making up p. They are equidistant from each
other, but there interaction creates a unique shape around the nucleus.
They are bilobed and can be visualized spread out along three axes.
Orbitals aid in our understanding of why some atoms
bond the way they do. For example carbon only has two free bonding
electrons (one in each p orbital), yet carbon forms four bonds to achieve
a higher stability. How is this actually accomplished. Carbon
promotes a 2s electron to a vacant p orbital and merges it s orbital with
its p orbitals creating a sp3 orbital that will accept four
electrons for bonding. The sp3 orbitals have slightly
higher energy than the 2s orbital and slightly lower energy than the p
orbital. This loss of energy though is more than made up by the higher
stability that can now be achieved via bonding.
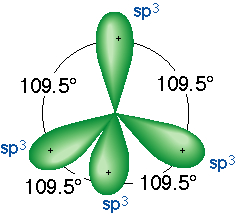
Note that each sp3 orbital is not bilobed.
Other atoms are capable of hybridizing their orbitals to create a variety
of unique orbitals that will allow increased stability through bonding.
This must be considered when deciding how atoms will bond. If higher
stability can be achieved, hybridization will likely occur.
Bonding occurs when orbitals from two atoms overlap
to the point that a stable electron arrangement occurs. If the bond
is a single bond between two of the same or different orbitals, it is called
a sigma bond. It occurs from the complete linear overlap of these
orbitals. Consider two s orbitals overlapping to create a sigma bond.
Consider two p orbitals forming a sigma bond.
If we consider CH4, we see that the sp3 orbitals
sigma bond to hydrogen atoms. In this diagram the overalpping shared
electrons are shown. Notice that their spins are opposite to each
other.
If molecules contain double or triple bonds, the first
bond formed will be a sigma bond, but this then restricts the orientation
of further bonding between orbitals. This results in a sideways overlapping
of orbitals creating what is called the pi bond. Due to this stretched
overlapping, the individual pi bond is always weaker than the sigma bond.
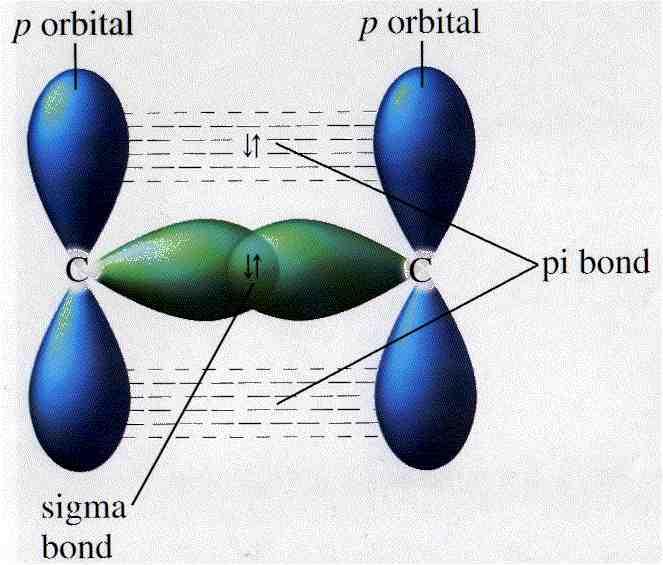
Consider the triple bond in the following molecule.
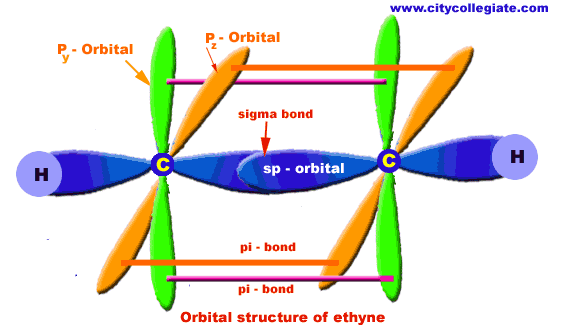
Molecular Shape
We know, then, what makes atoms stick together, but what limits
the proportions in which they can combine and what factors control molecular
shape? The simplest and most basic factor arises from the relative
sizes of the different atoms. The concept of atomic size is, however, somewhat
fuzzy: the size of an atom is controlled by the extent of its electron
charge cloud, and the density of electrons around an atom does not suddenly
reduce to zero; it gradually decays. Nevertheless it has proved possible
to assign approximate radii to atoms. Furthermore, purely geometrical
factors will limit the ways in which we can pack atoms together. These
factors become most obvious when we consider packing in crystals; thus
in crystal structures containing, for example, the large cesium ion, there
are often eight other atoms surrounding each cesium; whereas the smaller
lithium ion has room for only four.
The next point is that atoms have well defined combining
powers (the chemical concept of valence) which arises from specific features
of their electronic structure; in particular the number of electrons in
the outermost shell relative to the total number of electrons that can
be present in that shell. Indeed, the concept of valence springs
from one of the oldest and most powerful ideas of theoretical chemistry
—the 'electron pair' bond. Chemical bonds (like those between the two hydrogen
atoms in H2) often have a pair of associated electrons.
A key feature of chemical bonding to which we now turn concerns the fact
that it can have specific directional requirements in order to minimize
electron-electron repulsion within the molecules. This requires that
atoms rearrange themselves three dimensionally. These specific
geometrical requirements follow naturally from the criteria that the resulting
assembly of atoms should have the lowest possible energy. More specifically,
they can be understood in terms of the different shapes of the atomic electron
density charge clouds and by the ways in which these can interact.
Chemical bonding occurs where the atomic charge clouds interact and overlap
with each other. Therefore to determine the shape of a covalent
molecule, consider the number of electron pairs situated around the central
atom of the molecules and resolve the shape ot minimize the electron-electron
repulsion. However, if a lone electron pair is present, it will influence
the orientation of the other electron pairs but will not be realized in
the final identity of the molecule.
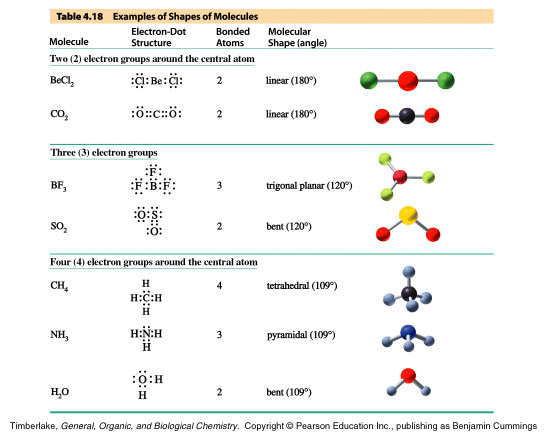
The process repeats with five electron pairs around
the central atom (due to hybridization). Here the main shape will
be the trigonal bipyramidal, but as lone pairs of electrons are included
the shape will be named differently as is the case for the tetrahedral
shape induced by four electron pairs in the chart above. As each
lone pair is added, the name changes as the shape is not fully visualized.
Molecular Polarity
There are, in fact, two sub-types of covalent bonds.
The H2 molecule is a good example of the first type of covalent
bond, the non-polar bond. Because both atoms in the H2
molecule have an equal attraction for electrons, the bonding electrons
are equally shared by the two atoms, and a non-polar covalent bond is formed.
Whenever two atoms of the same element bond together, a non-polar bond
is formed. A polar bond is formed when electrons are unequally shared
between two atoms. Polar covalent bonding occurs because one atom has a
stronger affinity for electrons than the other (yet not enough to pull
the electrons away completely and form an ion). In a polar covalent
bond, the bonding electrons will spend a greater amount of time around
the atom that has the stronger affinity for electrons. A good example of
a polar covalent bond is the hydrogen-oxygen bond in the water molecule.
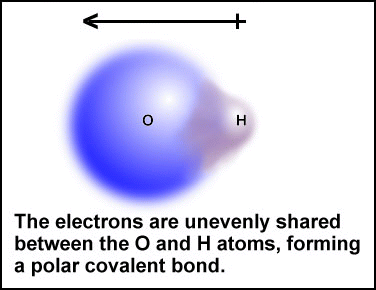
The primary difference between the H-O bond in water
and the H-H bond is the degree of electron sharing. The large oxygen atom
has a stronger affinity for electrons than the small hydrogen atoms. Because
oxygen has a stronger pull on the bonding electrons, it preoccupies their
time, and this leads to unequal sharing and the formation of a polar covalent
bond.
The ability of atoms to attract shared electrons
is termed electronegativity. Electronegativity values can be determined
by comparing atoms and this can be used to determine the type of bond that
would result. Trends do exist and for the most part they can be used
to predict bond types. Bond types actually exist as a continuum from
non-polar to ionic depending upon the relative strength of atoms involved.
An electronegativity difference of 0 indicates perfectly equal sharing,
but a value of 0-0.99 means unequal sharing. However, this unequal
sharing is not unequal enough to cause any significant polarity effects.
These bonds are still classified as nonpolar. If the electronegativity
difference between the two atoms exceeds 1.0, the unequal sharing is significant
enough to be detected and the bond formed is polar. If the unequal
sharing results in an electronegativity difference of greater than 1.7,
the sharing is so unequal that one atom rips an electron from the other
atom creating an ionic bonding. In fact, one would hardly call this
sharing at all. The ability to rip electrons away to create such
a clear unequal sharing of electrons can be determined by looking at %
ionic character of a bond. An electronegativity of 0 has a 0% ionic
character and the % increases from there until it is high enough to allow
ionic bonds to form.
Water molecules contain two hydrogen atoms bonded
to one oxygen atom (blue). Oxygen, with 6 valence electrons, needs
two additional electrons to complete its valence shell. Each hydrogen
contains one electron. Thus oxygen shares the electrons from two
hydrogen atoms to complete its own valence shell, and in return shares
two of its own electrons with each hydrogen, completing the H valence shells.
This means that water arranges its electron pairs to create a tetrahedron
around the oxygen atoms, but since there are only two bonds present, the
shape is bent. Since the OH bond is polar, water exists with a discrete
partial negative region and a partial positive region within the neutral
molecule. This is due to the unequal distribution of electrons.
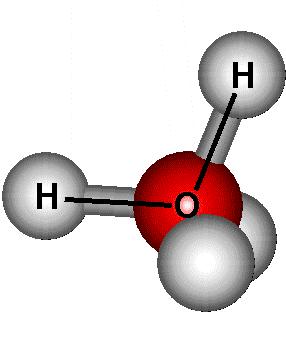 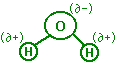 
Because the valence electrons in the water molecule
spend more time around the oxygen atom than the hydrogen atoms, the oxygen
end of the molecule develops a partial negative charge (because of the
negative charge on the electrons). For the same reason, the hydrogen
end of the molecule develops a partial positive charge. Ions are
not formed, however the molecule develops a partial electrical charge across
it called a dipole. This allows water to orientate itself in different
manners depending up whether a positive or a negative charge is nearby.
A nonpolar molecule may have polar bonds but it will always orient itself
the same way in response to nearby charges.
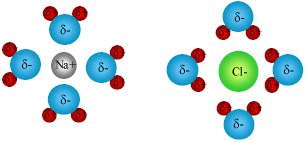
|